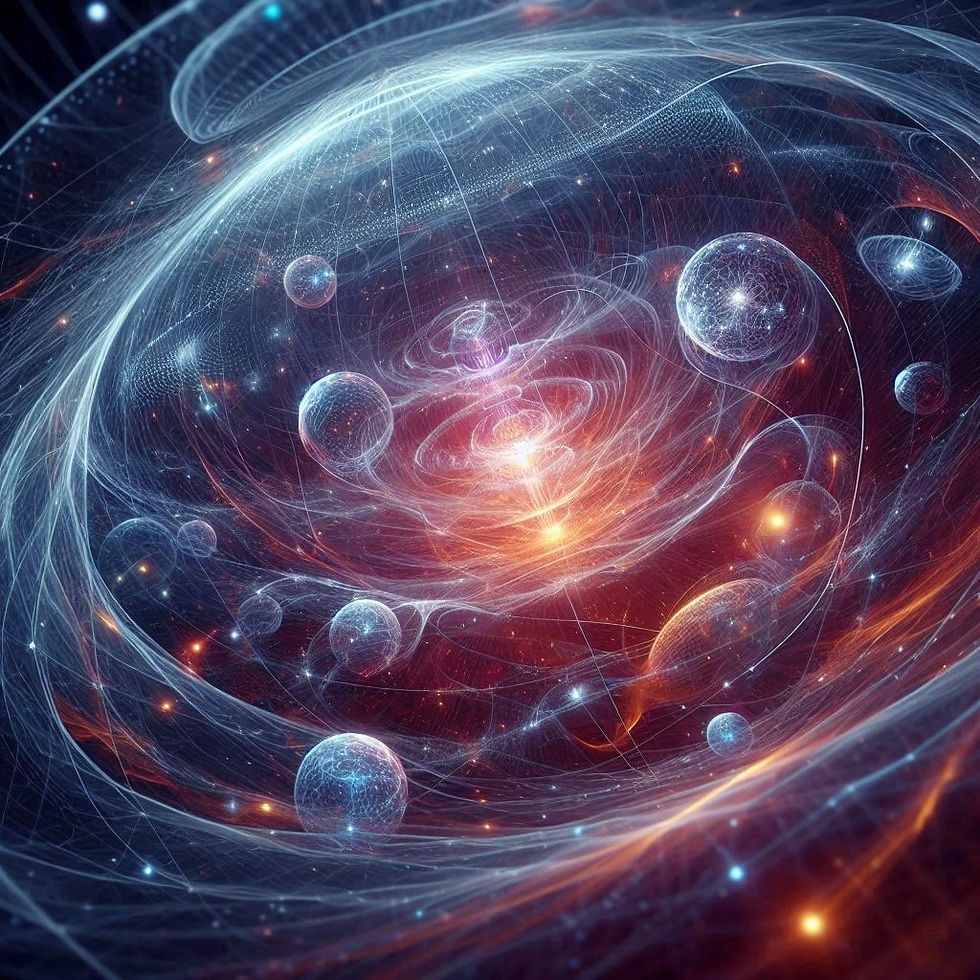
The universe is not just made of particles and forces but also filled with invisible fields that shape reality at every level. These fields extend through space and interact with particles, governing everything from gravity and electromagnetism to the very nature of mass. While they cannot be directly observed, their effects can be measured, making them one of the most fundamental aspects of modern physics.
Fields serve as the underlying framework that dictates how forces work, how particles acquire mass, and how the universe evolves. Each fundamental force has an associated field, and quantum mechanics reveals that even particles themselves are excitations or disturbances within these fields.
Analyzing Fields: The Invisible Forces of Nature
Fields permeate space and dictate the interactions between objects, governing everything from planetary orbits to the smallest subatomic processes.
The Gravitational Field: The Warping of Spacetime
Gravity is the fundamental force responsible for the attraction between masses, governing the motion of planets, stars, and galaxies. It is the reason why objects fall to the ground on Earth and why celestial bodies remain in orbit around one another. The gravitational field is an invisible yet ever-present influence that extends throughout the universe, affecting everything from the largest cosmic structures to the bending of light itself.
Historically, Newtonian mechanics described gravity as a force that acts at a distance between two masses. According to Isaac Newton’s Law of Universal Gravitation, every object in the universe attracts every other object with a force that depends on their masses and the distance between them. This model successfully explained planetary motion, predicting how celestial bodies interact in space and why objects fall toward the ground. However, it could not fully account for certain observed phenomena, such as the precise orbit of Mercury or the bending of light around massive objects.
A deeper understanding of gravity emerged with Einstein’s General Theory of Relativity, which replaced Newton’s idea of a gravitational "force" with the concept of spacetime curvature. According to Einstein, gravity is not an invisible force pulling objects together but rather the result of massive objects warping the fabric of spacetime itself. This curvature dictates the motion of objects, causing them to follow curved paths that we perceive as gravitational attraction. The greater the mass of an object, the more it distorts spacetime, leading to stronger gravitational effects.
This new perspective on gravity explains several key phenomena. One such effect is gravitational lensing, where light from distant stars or galaxies bends as it passes near massive objects like black holes or galaxy clusters. Another consequence is time dilation, where time runs slower in stronger gravitational fields—an effect observed near black holes and confirmed through experiments with precise atomic clocks. Additionally, Einstein’s theory refined our understanding of planetary motion, accurately predicting anomalies in Mercury’s orbit that Newtonian mechanics could not fully explain.
The gravitational field extends infinitely, influencing objects across vast cosmic distances. However, its strength diminishes with distance, meaning that its effects become weaker as objects move farther apart. Unlike other fundamental forces, such as electromagnetism, which has both attractive and repulsive components due to positive and negative charges, gravity has no known "opposite" force. It is always attractive and acts universally on all forms of mass and energy. This uniqueness makes gravity fundamentally different from the other forces governing the universe.
Despite our deep understanding of gravity, mysteries remain. Scientists continue to search for a quantum theory of gravity that would reconcile General Relativity with quantum mechanics. The nature of dark matter and dark energy, which appear to influence gravity on cosmic scales, also remains an open question. Nonetheless, the gravitational field remains one of the most crucial and mysterious aspects of the universe, shaping the very fabric of reality.
The Electromagnetic Field: The Force Behind Light and Charge
The electromagnetic field is one of the fundamental fields of nature, governing the interactions between charged particles. It is responsible for both electric and magnetic forces, which together shape a vast range of physical phenomena, from the behavior of atoms to the transmission of light across the universe. Unlike gravity, which only attracts, the electromagnetic force can both attract and repel, making it one of the most versatile and influential forces in physics.
This field is mathematically described by Maxwell’s equations, a set of four fundamental equations formulated by James Clerk Maxwell in the 19th century. These equations unified electricity and magnetism into a single framework, demonstrating that electric and magnetic fields are intrinsically linked. When an electric charge moves, it generates a magnetic field, and a changing magnetic field, in turn, induces an electric field. This interplay between electricity and magnetism explains everything from how electric currents flow in circuits to how magnets create force fields.
The electromagnetic field plays a crucial role in multiple physical processes:
Electricity – The movement of charged particles, such as electrons, creates electric currents. This is the basis for electrical circuits, power generation, and countless technological applications.
Magnetism – Moving charges generate magnetic fields, which in turn can influence other charges and currents. This principle is the foundation of electromagnets, electric motors, and generators.
Light – Perhaps the most remarkable feature of the electromagnetic field is that it gives rise to electromagnetic waves, which include visible light, radio waves, X-rays, and gamma rays. These waves do not require a medium to travel and can move through the vacuum of space at a constant speed—the speed of light (c ≈ 299,792,458 m/s).
The discovery that light itself is an electromagnetic wave was one of the greatest breakthroughs in physics. Maxwell’s equations predicted the existence of self-sustaining electromagnetic waves, which were later confirmed experimentally. These waves make up the electromagnetic spectrum, which includes not only visible light but also radio waves (used in communication), microwaves (used in cooking and radar), infrared radiation (felt as heat), ultraviolet light (which causes sunburn), X-rays (used in medical imaging), and gamma rays (emitted in nuclear reactions).
One of the unique properties of the electromagnetic force is that it has both attractive and repulsive interactions. Unlike gravity, which always pulls objects together, electromagnetic forces depend on the charge of particles. Opposite charges (positive and negative) attract, while like charges repel. This repulsion is what prevents electrons from collapsing into the atomic nucleus and plays a key role in chemical bonding, material properties, and even biological processes.
The electromagnetic field is essential to our understanding of nature and has countless applications in modern technology. It enables electricity, communication systems, medical imaging, and even quantum mechanics. Without it, life as we know it would not exist, as electromagnetic interactions are responsible for holding atoms and molecules together. As research continues, scientists are exploring deeper questions about electromagnetism, including its role in quantum field theory and its unification with other fundamental forces of nature.
Quantum Fields: The Foundation of Particle Physics
In modern physics, Quantum Field Theory (QFT) provides the most accurate and fundamental description of reality. It revolutionized our understanding of matter and energy by proposing that what we perceive as individual particles—such as electrons, quarks, and photons—are not isolated objects but rather disturbances or excitations in underlying quantum fields. These fields permeate all of space, and particles emerge from them as localized waves or ripples.
Each fundamental particle in nature is associated with its own quantum field. For instance, the electron field governs electrons, determining their behavior and interactions. Similarly, the photon field is responsible for electromagnetic interactions, as photons are the force carriers of light and electromagnetism. The quark field governs quarks, which are the fundamental building blocks of protons and neutrons, forming the basis of atomic nuclei. Instead of viewing particles as tiny, discrete objects moving through space, QFT suggests that space itself is filled with fluctuating fields, and particles appear whenever these fields are excited.
This concept has profound implications for understanding quantum mechanics and the fundamental nature of the universe. One of the most striking consequences of QFT is the existence of virtual particles, which can momentarily appear and disappear in empty space. This phenomenon, known as quantum fluctuations, plays a crucial role in particle interactions and even influences the structure of space at microscopic scales. Another fascinating aspect of quantum fields is quantum entanglement, where two or more particles become instantaneously connected, regardless of the distance between them. This bizarre property has been experimentally confirmed and challenges our classical understanding of locality and causality.
Additionally, QFT introduces the idea that reality is inherently probabilistic rather than deterministic. Unlike classical physics, where an object's future behavior can be predicted with certainty given enough information, quantum fields operate according to probability distributions. This means that events at the quantum level do not have definite outcomes but instead follow statistical patterns, as described by equations such as the Schrödinger equation and Feynman’s path integral formulation.
Quantum Field Theory remains one of the most successful theories in physics, forming the foundation of the Standard Model, which describes all known elementary particles and forces (except gravity). However, challenges remain, particularly in unifying QFT with General Relativity to develop a complete theory of quantum gravity. Despite these open questions, quantum fields continue to be at the heart of our understanding of the subatomic world, offering profound insights into the very fabric of reality.
The Higgs Field: The Origin of Mass
The Higgs field is a special quantum field that plays a crucial role in the universe by giving mass to fundamental particles. In the framework of the Standard Model of Particle Physics, particles were originally thought to be massless. However, experiments revealed that particles like electrons, quarks, and others do, in fact, possess mass. This discrepancy created a puzzle for physicists, as the Standard Model did not explain how these particles acquired mass. The solution came with the discovery of the Higgs field and its associated particle, the Higgs boson, which was confirmed in 2012 through experiments at CERN's Large Hadron Collider.
The Higgs mechanism describes the process by which mass is generated. According to this theory, space is filled with the Higgs field, and as fundamental particles move through it, they interact with this field. The strength of the interaction between a particle and the Higgs field determines how much mass that particle acquires. Some particles interact strongly with the Higgs field and acquire significant mass, while others interact weakly or not at all and remain massless.
For example, photons, the particles of light, do not interact with the Higgs field and, as a result, remain massless. On the other hand, quarks and electrons, which interact more strongly with the Higgs field, acquire substantial mass. This difference in interaction explains why some particles, such as the building blocks of matter, have mass while others, like photons, do not.
Without the Higgs field, the universe as we know it would not exist. Without mass, particles would not be able to form atoms, which are the building blocks of matter. If particles like electrons had no mass, they would not be bound to atomic nuclei, and the complex chemistry that leads to the formation of stars, planets, and life would be impossible. The Higgs field, therefore, is essential for the creation of the matter that makes up the universe. Its discovery marked a significant milestone in physics, confirming one of the key predictions of the Standard Model and deepening our understanding of the fundamental forces that shape the universe.
In essence, the Higgs field is not just a theoretical concept but a fundamental ingredient that enables the universe to have structure and the possibility of life. Without it, the world would be an entirely different place, with particles moving through space without the mass needed to form the complex structures we see today.
Why Fields Matter
Fields are not merely abstract or theoretical constructs; they are the very foundation upon which the universe operates. They shape the behavior of all physical phenomena and govern the interactions between forces, particles, and energy across space and time. In essence, fields are the medium through which forces act, and they provide the structure for understanding the nature of matter and the laws that govern its behavior.
At a fundamental level, fields determine how forces interact. The electromagnetic field governs the interactions between charged particles, leading to phenomena like electricity, magnetism, and the propagation of light. Similarly, the gravitational field dictates how mass and energy influence spacetime, leading to the attraction of objects and the bending of light around massive bodies. These fields underpin the four fundamental forces of nature: gravity, electromagnetism, the strong nuclear force, and the weak nuclear force. Understanding how fields work is essential for understanding how forces interact in nature, from the smallest particles to the largest galaxies.
One of the most profound aspects of fields is their role in explaining why particles have mass. Without the Higgs field, fundamental particles would remain massless, and the universe would be an entirely different place. The Higgs mechanism, which is a process by which particles acquire mass through their interaction with the Higgs field, explains why some particles, like quarks and electrons, have mass, while others, like photons, remain massless. This interaction is vital for the formation of atoms and the complex structures that allow for life as we know it. Without this mechanism, the universe would lack the building blocks necessary for atoms and molecules to form, making the existence of matter—and thus life—impossible.
Fields also form the foundation of quantum physics, which is the branch of physics that describes the behavior of particles at the smallest scales. Quantum Field Theory (QFT) treats particles not as discrete objects but as excitations or disturbances in underlying quantum fields. This revolutionary perspective transforms our understanding of the nature of reality, suggesting that the entire universe is filled with energy and that particles emerge as localized disturbances within these fields. Quantum fields help explain phenomena like quantum entanglement, where particles appear to be instantaneously connected across vast distances, and the probabilistic nature of quantum mechanics, where particles do not have definite properties until they are measured.
Although fields are invisible and often intangible in our daily experience, their effects are felt throughout the universe. Whether it’s the force that keeps us grounded on Earth through gravity, the way electricity powers our homes, or the subatomic interactions that drive the formation of atoms, fields shape the universe at every scale. They influence everything from the vast expanse of galaxies to the interactions between the tiniest particles inside atoms. Fields are central to understanding the nature of space, time, and matter, and they provide the framework for modern physics.
Would you like to explore any specific field in more detail, such as quantum field theory, which underpins our understanding of particle physics, or gravitational waves, which offer insight into the ripples in spacetime caused by massive objects like black holes or neutron stars? Both fields are crucial to understanding the universe at its most fundamental level, and delving into them can offer fascinating insights into the nature of reality.
References:
Einstein, A. (1915). Die Feldgleichungen der Gravitation [The Field Equations of Gravitation]. Sitzungsberichte der Preußischen Akademie der Wissenschaften.
Feynman, R. P. (1965). The Feynman Lectures on Physics. Addison-Wesley.
Higgs, P. W. (1964). Broken Symmetries and the Mass of Gauge Bosons. Physical Review Letters, 13(16), 508–509.
Maxwell, J. C. (1865). A Dynamical Theory of the Electromagnetic Field. Philosophical Transactions of the Royal Society of London, 155, 459–512.
Newton, I. (1687). Philosophiæ Naturalis Principia Mathematica [Mathematical Principles of Natural Philosophy]. Royal Society of London.
Planck, M. (1900). Zur Theorie des Gesetzes der Energieverteilung im Normalen Spektrum. Verhandlungen der Deutschen Physikalischen Gesellschaft, 2, 237-245.
Weinberg, S. (1995). The Quantum Theory of Fields. Cambridge University Press.
Commenti